
GAS GIANT INTERIORS
What are the outer gas giants made of? depend who you ask.
I became interested in the outer gas giants several years ago. Everything I had known from my previous scientific work, and especially my experience from researching comets (see more here) and dwarf planets (see more here) had taught me that the icy objects that roam in the outer Solar system are actually not that icy at all. Yes, they are definitely capable of retaining ice, because they are hanging around out there in the coldest regions of our Solar system where many different ices can remain frozen. However, it turns out they are actually composed mostly of refractory materials, which are materials that cannot sublimate easily like volatiles do. Rocky minerals and various organic compounds contribute (as an approximate rule of thumb) about 3/4 of the mass of small outer solar system bodies, while the mass fraction of ices is much smaller, around 1/4. In other words, the rock/ice mass ratio in these objects is at least 3.
So the first thing that interested me about the outer giants Uranus and Neptune is why they are called 'icy giants'. The official reason is because they are supposed to be composed from about twice as much water as rock, given classic internal composition models. And since water is an ice, being volatile under the conditions we are used to in our ordinary lives, Uranus and Neptune are called icy giants. But hold on... planets grow by accreting mass from their surroundings. And if the solids they accreted early-on were actually ice-poor, how is it possible that Uranus and Neptune are ice-rich?
​
This composition tension between the outer giants and their building blocks gave myself and additional collaborators ample motivation to carry out further research together. What might the solution be? There are actually only two possibilities -- either Uranus and Neptune are not icy at all, or there is a way to assemble an icy planet from ice-poor building blocks.
​
For the first couple of years we have tried to investigate the first option. If Uranus and Neptune aren't dominated by water, and in fact are rock-rich, is it possible that people have been wrongly calling them ice giants all these years? Are they actually rock giants instead? It turns out there is a way to check.
​
Planets do not have uniform densities. Their densities change with increasing depth, just like the Earth has a denser core underlying a less dense mantle, underlying a less dense crust. What we definitely know about these planets is their radius, mass and something about how their mass is internally distributed. The first two we know with greater accuracy. The third is called the moment of inertia and it is associated with a bit more uncertainty. In order to figure out what the planet is like on the inside, we can first mathematically link these three quantities to specific density profiles. However, it is important to understand that the number of possible density profiles we can find is essentially limitless. Given the same set of these three quantities, one can find a particular density profile which is relatively flat, or an equally good density profile which has a smaller but denser core. There are infinite possibilities that work mathematically. Unlike on the Earth, we do not have seismic information that helps us choose. In collaboration with Professor Morris Podolak of Tel-Aviv University, our approach has therefore been to map the entire set of possibilities! We let the computer randomly draw hundreds of thousands of different density profiles, and as long as they are mathematically commensurate with the radius, mass and moment of inertia, they are all acceptable. Next we use an algorithm to match these density profiles with corresponding pressure, temperature and composition profiles, giving us complete models of the interior of the planet. The composition is particularly challenging to obtain. One has to decide which materials are included, and to know the relation between the density of this material and every possible combination of the pressure and temperature it might experience inside the planet. This relation is called an equation of state. Luckily, we have access to such equations of state, because they are commonly used by scientists who investigate the inner structure of planets. We used equations of state for iron, silica (a type of common rock mineral composed of one silicon and two oxygen atoms), water and a gas mixture of hydrogen and helium that makes up the outermost atmosphere (alike the composition of the Sun), in similarity to most other studies. We later also investigated the materials methane (composed of one carbon and four hydrogen atoms) and carbon monoxide (composed of one carbon and one oxygen atom). For the latter, we first had to invent a new equation of state, because a proper one did not exist in the relevant literature.
​
Using this elaborate code, we essentially ask the computer to find out for us: "what possible compositions can planets have?". The input to the code is the planet's mass, radius and moment of inertia, and the output of the code is a detailed array of physically allowed models. We cannot know which one of these random models is more likely than the other, but we can find out what 'limits' exist in the phase space of possibilities. Using our approach, we discovered that for Uranus and Neptune the rock/water mass ratio is limited to a value between 1-2. There is only one way to exceed this limit, in theory, and it involves mixing of the rock or iron within the planet with a large fraction of hydrogen. However, it is not yet clear whether that is physically possible or likely to happen during the planet's formation. Setting aside this seemingly dubious option, our best efforts to transform Uranus and Neptune to rock giants, with a rock/water mass ratio of 3 or more, have come up empty.
​
But that's not the end of the story, since there was still one remaining path to an alternative solution - creating an icy planet from ice-poor building blocks. That may seem impossible, but then an idea came up. Half a century ago researchers briefly discussed the idea that chemistry could alter carbon monoxide by turning it into methane and water. We decided to revive and greatly extend this idea.
​
In the contemporary Solar system, carbon monoxide is not a particularly abundant material. While we do know that comets can incorporate carbon monoxide, it is usually a negligible constituent, and we think it typically only exist as trapped gas inside other amorphous ices or perhaps as pure ice condensates near the cold surface of particularly distant comets (read about it here). Therefore, even if chemistry were to be invoked, carbon monoxide in itself would not do much to alter the composition of the planet. However, unlike 50 years ago, decades of research have revealed that comets actually harbor vast amounts of carbon, not inside ices, but inside refractory organic materials. New evidence suggest that up to about 50% of their mass could consist of these refractory organics. Hence, comets actually contain a lot of carbon that may be readily transformed through chemistry.
​
Using several steps, we showed that this transformation can occur, and lead to the formation of copious amounts of methane during the planet's growth. We first use a complex computer model developed by collaborator Professor Peter Bodenheimer from UCSC, to simulate Uranus' growth, revealing the details of its atmospheric density, pressure and temperature conditions at different points in time. We then use a different computer code that tracks the passage of comet-like solids of various sizes, that are accreting in the atmosphere during growth, increasing the planet's mass. This second code takes as input the output from the first code, and tells us exactly where in the atmosphere these solids dissolve. The calculation involves frictional and thermal heating, as well as ram pressure. Now that we have the location and pressure-temperature conditions experienced by the dissolved material from the second code, we can finally decide what the result of chemistry leads to. For this we use yet a third code that determines the chemistry.
​
Although there are complications, and future work would have to address details that are currently beyond the level of any existing model within our scientific community, our preliminary results do indicate that as the planet grows, large amounts of methane can form. In fact, we find that the upper limit of methane mass fraction in the planet could be between about 20-45%, making Uranus and Neptune methane giants. Different assumptions regarding the exact composition of the comet-like solids are responsible for spanning the aforementioned range.
​
When we use our random model code to generate interiors with methane, and we compare the phase space of possible compositions to the results of our chemical calculations, we discover an excellent fit:
​
​
​
​
​
​
​
​
​
​
​
​
​
​
The figure above depicts this match. Each sub-plot (Uranus-left / Neptune-right) shows the phase space of possible compositions, as each random model generated by the computer is indicated by a single pixel. The Y-axis shown here is the methane fraction, as a function of the water/rock (here iron and silica together are considered rock) mass fraction inside the planet. The computer is showing us what is the allowed space of possibilities, given the radius, mass and moment of inertia of the planet. The color coding shows the temperature at the center of the planet, a value which has some physical significance. The red asterisks, however, are models that were produced from the aforementioned chemical calculation, and one can see that they independently match the spatial distribution in the phase space of random models, so they adhere to the same physical limitations.
​
What does it mean if Uranus and Neptune are methane giants? And could other giants like Saturn and Jupiter also be methane rich? The answer to the first question is that if the outer giants turn out to be methane rich, it is a completely new paradigm, and one would have to account for it when considering models of their formation, evolution, impacts, etc. However, this study is only the first stepping stone to further theoretical work which is still required in order to verify our ideas.
Our model also offers a prediction. Since atmospheric hydrogen participated in making methane, the current hydrogen/helium ratio in the bulk atmosphere should be considerably lower than the Solar ratio. Upcoming missions to Uranus could, hopefully, either validate or falsify this prediction. It should also be noted that exo-solar planets that are similar to Uranus and Neptune in mass, could likewise be methane-rich. The mechanisms we suggested should be universal.
​
The answer to the second question is that Saturn and Jupiter are definitely not methane-rich. There are two reasons. The first is that, being more massive, the pressure conditions inside them will lead to the dissociation of methane (into carbon and hydrogen separately). Besides, there is so much hydrogen and helium in Saturn and Jupiter, that their envelope gas fraction actually dwarfs the relative fraction all other materials heavier than helium, combined.
​
Future investigation is certainly required in order to improve both the random modeling aspects as well as the chemical calculations, and we hope to continue this work in the coming years.
​
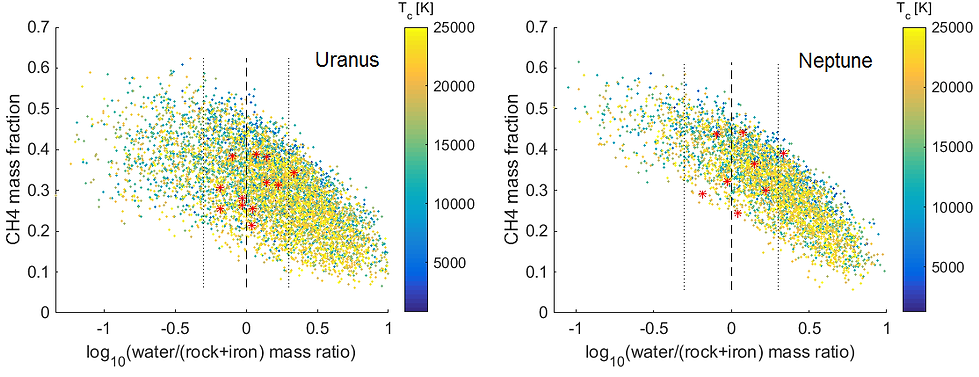